Gravitational lensing
According to Albert Einstein's theory of general relativity, massive astrophysical objects, such as galaxies or galaxy clusters, distort the spacetime they are embedded in. As light travels through spacetime, it is deflected by this distortion and its path will be curved. This effect is called gravitational lensing. If there is a distant light source behind the massive object, “the lens”, and along our line of sight, multiple distorted images of the background object could form, sometimes even into a complete ring, called an Einstein ring.
You can see a sketch of this phenomenon in the image below, with a background galaxy as the light source (in red) whose light paths are distorted by a foreground galaxy. The observed image of this particular setup is shown on the right.
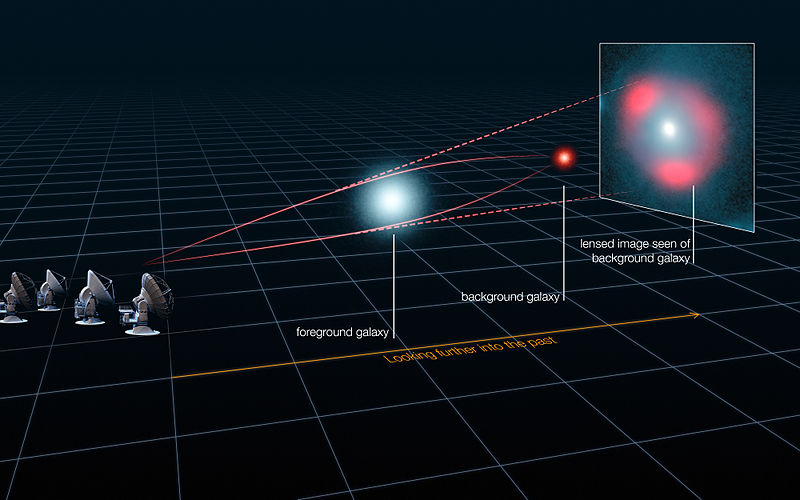
We discriminate between four different types of lensing:
- Strong lensing: if the background source is very well aligned with the foreground lens, multiple distorted and magnified images can be resolved. The sketch above shows a galaxy-galaxy strong lensing system. The image below shows strong lensing by a foreground cluster which is a more complex setup.
- Weak lensing: when the background source is far off the line of sight, only one distorted image is visible. The image below also shows weak lensing features as slightly elongated galaxies far from the cluster center.
- Millilensing: additional deflections of the background source light can also be caused by structures within the foreground lens, such as dark matter satellites and local mass density perturbations.
- Microlensing: small distortions of a lensed image by the individual stars of the foreground lens causing additional (de-)magnification; As it creates no resolvable images it is not visible in the image below.
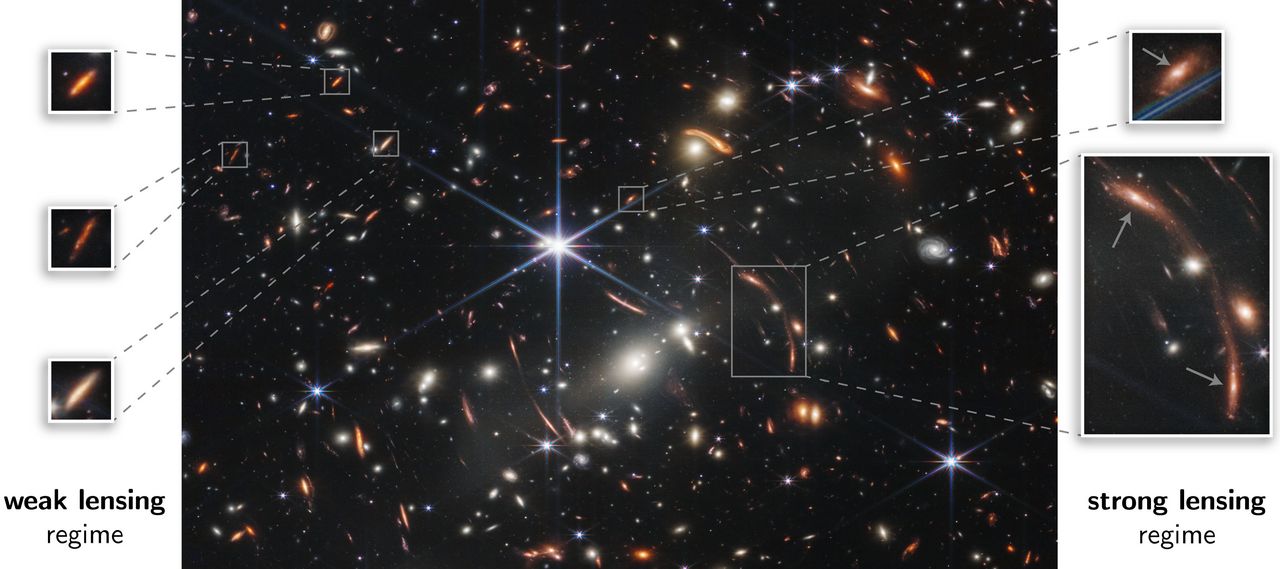
Gravitational lensing can be used to tackle many different astrophysical questions, as it involves various objects at different cosmological distances. Find out more below about the fields our group investigates.
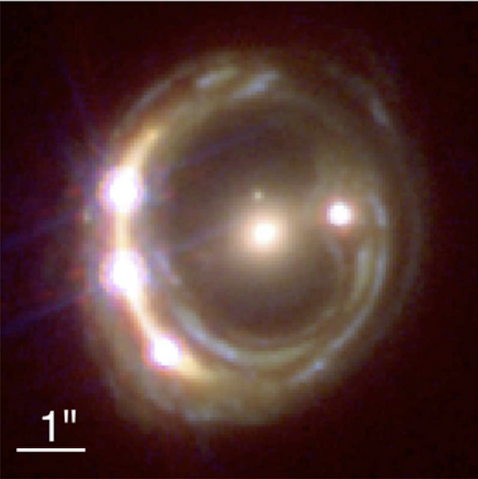
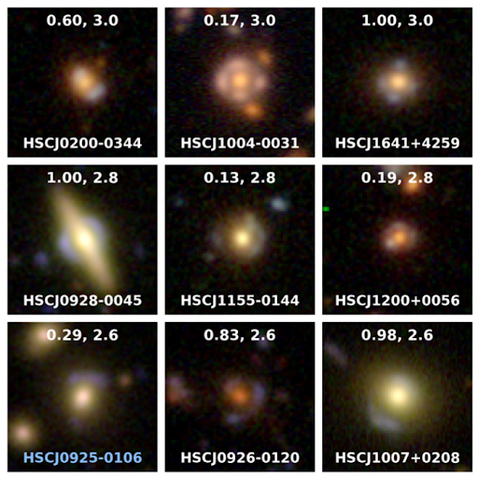
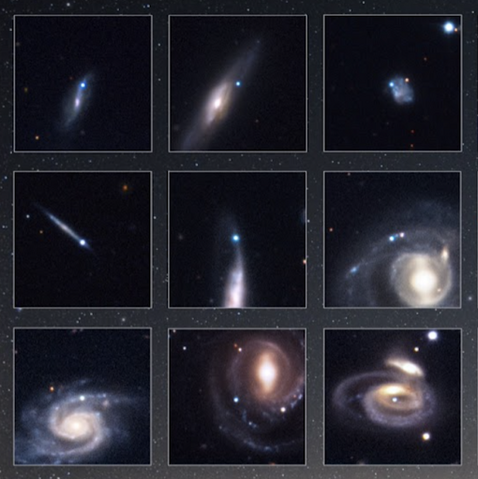
One of the greatest discoveries in cosmology is that our Universe is expanding. Georges Lemaître and Edwin Hubble independently found that galaxies that are further away from us are receding from us as a faster speed - the proportionality constant between the speed and distance is known as the Hubble constant (H0). The Hubble constant is a key cosmological parameter that sets the expansion rate of the Universe. Given its importance, various methods have been developed over the past decades to measure its value.
As the precisions of the H0 measurements are improved in recent years, an intriguing tension in the H0 measurements between some of the methods has emerged. In particular, there is a ~5 sigma tension between the measurements from the Cepheid distance ladder of the SH0ES program (Riess et al. 2022) and the cosmic microwave background observations (Planck collaboration 2020). This tension, if verified, implies that new physics beyond the standard cosmological model “flat ΛCDM” is required. Therefore, independent methods are crucial to assess the significance of the tension.
In our research group, we employ two independent and competitive methods to measure H0: (1) strong lensing time delays, and (2) supernovae expanding photospheres.
Strong gravitational lens time delays
We have measured H0 = 73.3+1.7/-1.8 km/s/Mpc (with 2.4% precision) in flat ΛCDM using a sample of 6 lensed quasars with physically motivated lens mass models as part of the H0LiCOW, COSMOGRAIL, SHARP and STRIDES programs, now collectively known as the TDCOSMO collaboration (Wong et al. 2020, Shajib et al. 2020, Millon et al. 2020). We have further explored the robustness of the results by relaxing the assumptions on the mass model (Birrer et al. 2020). In this context, we have demonstrated that spatially-resolved (2D) kinematics of the lens galaxies are crucial to constrain the lens mass models for accurate and precise H0 determinations, and have developed state-of-the-art lensing and dynamical modeling software (Yıldırım et al. 2020; submitted; Shajib et al. 2023). We have also automated the lens mass modeling procedure to increase the efficiency of our analysis (Ertl et al. 2023). We successfully led a JWST proposal and were granted time in cycle 1 (PI: Suyu) to observe a lensed quasar system (Figure 1) and obtain its 2D kinematics.
By combining lensing and supernova expertise at MPA and TUM, our research group has launched a new program on lensed supernovae: HOLISMOKES! The program is funded by the European Research Council and aims to use lensed supernovae (SNe) instead of lensed quasars for measuring H0. We are developing new techniques to find the much rarer lensed SNe, to model quickly their mass distribution, investigate impact of microlensing on SNe, and measure their time delays (see HOLISMOKES Publications). Figure 2 below shows examples of new candidate lens systems that we discovered (Papers II, VI, VIII) and monitor daily with the ZTF alert stream to find lensed SNe.
Supernova expanding photospheres
Together with colleagues at ESO, MPA and TUM, we have initiated the adH0cc program to measure distances in the local universe by modeling the expanding atmospheres of Type IIP SNe (EPM - Expanding Photosphere Method). The project can be split into two parts: the acquisition and careful calibration of a tailored data set of Type IIP SNe in the nearby Hubble flow (0.03 < z < 0.10), and the development of the radiative-transfer code and further refinement of the modeling techniques to determine EPM distances from these data.
We have obtained high-quality spectroscopic and photometric time series for 21 SNe IIP in the targeted redshift range through an ESO Large Programme (PI: Leibundgut). This is more than our initial goal and will allow us to check for systematics within our data set that arise from different properties of the SNe or their environments. We show in Figure 3 below a gallery of some of the new SNe IIP that we have observed.
Significant improvements have also been achieved on the modeling side. Non-local-thermodynamic-equilibrium effects and continuum processes have been included in the radiative-transfer simulations, and the actual spectral fitting is now accomplished by an emulator that has been trained on a multi-dimensional grid of simulated spectra, thus speeding up the fitting process by several orders of magnitude and allowing for an automated maximum-likelihood fitting procedure.
Quasars are among the brightest sources in the universe, powered by the accretion of matter onto supermassive black holes. In the high-redshift frontier, these sources are prime laboratories for understanding the formation, growth, and structure of the first black holes and galaxies. It is widely known that the black hole mass often correlates with the mass of the bulge, luminosity, and velocity dispersion of its host galaxy. The origin of these relations, however, is not fully understood yet. Mapping these correlations to higher redshifts therefore helps to decipher the origin of these relations and to understand the co-evolution of the black holes and their host galaxies.
In the high-redshift universe, hundreds of quasars have been discovered. Interestingly, about ten percent of them belong to a class called ‘‘young quasars’’ showing lifetimes of only a few thousand years. These young quasars pose problematic issues since their ages are many times shorter than the typical timescale required for growing supermassive black holes – i.e., a few million to billion years. Additionally, a notable subset of high-redshift quasars exhibits weak emission lines, in contrast with the general quasar population. Therefore, expanding the sample size at this early era is crucial for investigating the connection between the rare weak-line sources and the cases of very young quasars, which might provide insights into the early growth modes (e.g., Andika et al., 2020, 2022a).
Another prominent issue in the field of high-redshift quasar searches is the rarity of the gravitationally lensed sources (examples of lensed quasars shown in Figure 1). Because of the large lensing optical depths attained at this distance, it has been anticipated for decades that up to one-third of the high-redshift quasars will be strongly lensed. A re-examination of the search strategies employed in the past quasar surveys reveals a significant selection bias against lensed quasars. Accordingly, constructing a more robust selection method to uncover such hidden populations of lenses will be a crucial experiment to conduct (e.g., Andika et al. 2022b).
With the help of gravitational lensing, we are able to probe some quasars with remarkable clarity – thanks to flux magnification and, very importantly, an increased effective spatial resolution – and observe their inner region in detail. In particular, the physical parameters of the supermassive black hole have been studied: (i) high-resolution images have helped us to obtain the total and bulge luminosity of the host galaxy (Ding et al. 2017; Figure 2), (ii) medium-resolution spectrograph has helped us to obtain the black hole mass of lensed quasars (Melo et al. 2021, Melo et al. in prep. I), and the accretion disk size.
Therefore, strong gravitationally lensed quasars are notable cosmic probes to investigate the origin of black hole/galaxy co-evolution, especially in the low-luminosity regimes. By utilizing cutting-edge observational techniques, including high-resolution imaging and spectroscopy, and advanced computational models, we aim to shed light on the astrophysical properties of these fascinating objects.
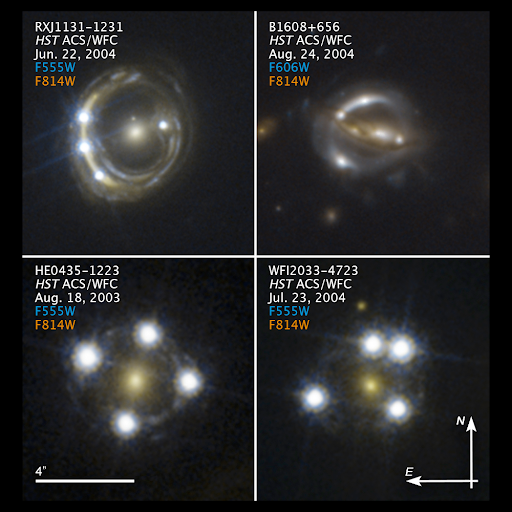
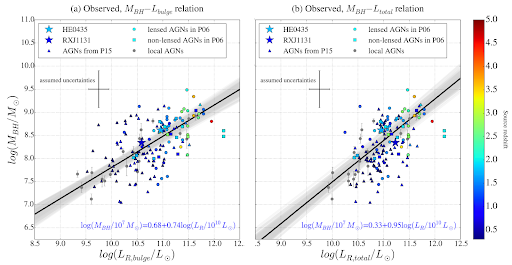
Galaxy clusters are the most massive, gravitationally cohesive structures of the Universe, making them the building blocks of the large scale of the Universe. Furthermore, the well established scenario of hierarchical structure formation predicts that small overdensities undergo merging events, growing in mass across cosmic time and forming massive clusters of galaxies. In such a scenario, clusters act as crossroads between cosmology and astrophysics and thus carry precious cosmological and astrophysical information. Their abundance is mainly driven by the amplitude of mass density fluctuations and the total mass density of the Universe, Ωm.
With the mass distribution composed mainly of dark matter (~ 90%), galaxy clusters are excellent laboratories for studying cosmology and physical processes related to structure formation and evolution. Thanks to gravitational lensing (see Figure 1 as example), we can obtain accurate measurements of the total mass distribution of galaxy clusters out to 1 mega-parsec with much higher precision than other methods, such as dynamics and X-ray emission. Such measurements of the mass distribution in clusters show that the cosmological model ΛCDM still has some issues in reproducing all observations in these scales.
On the other hand, we can also use the large number of multiply lensed background galaxies to probe the background geometry of the Universe, i.e. quantities such as Dark Energy and its global properties, as illustrated in Figure 2. Galaxy cluster strong lensing cosmography is a promising method with great synergy with other observables, such as type Ia supernova and cosmic microwave background.
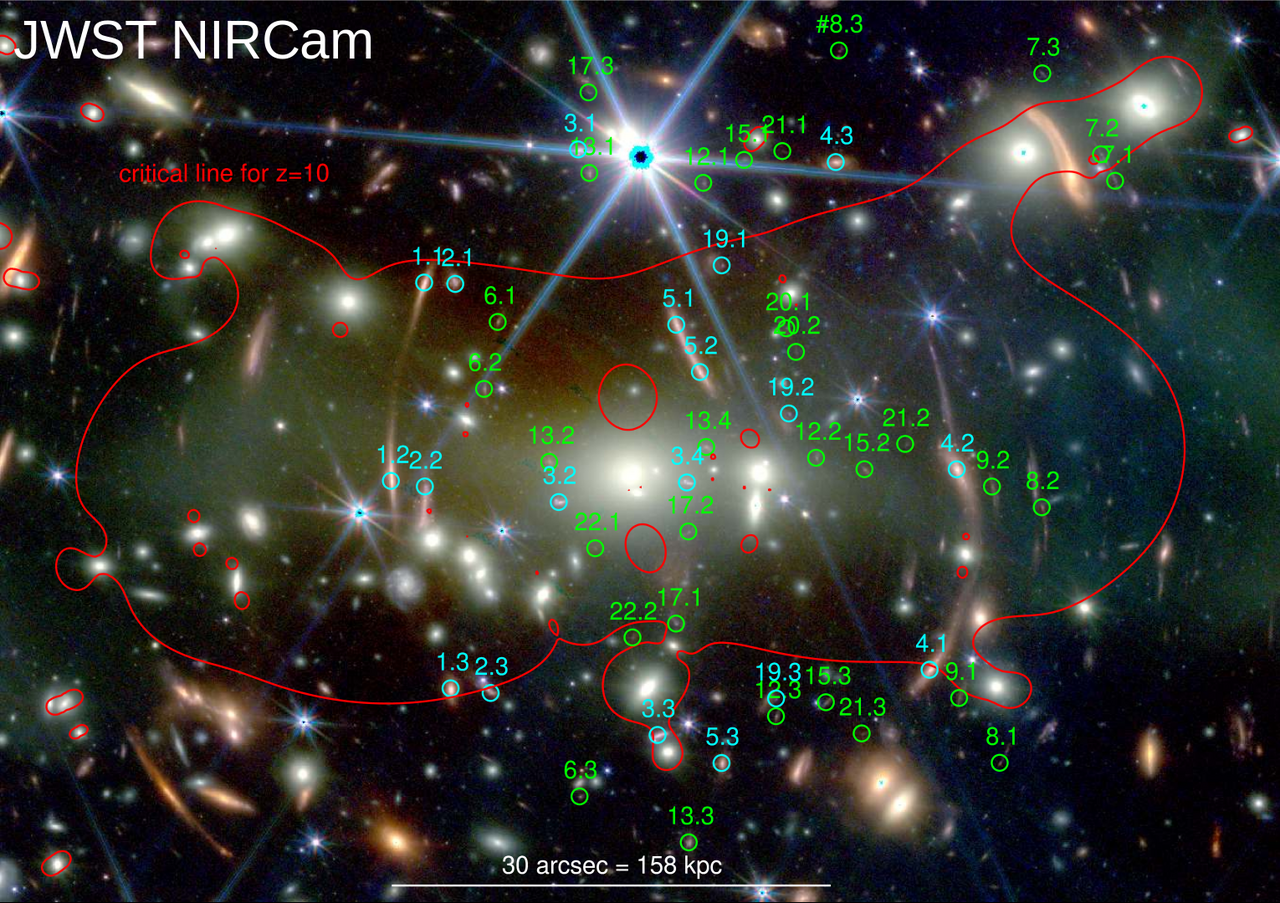
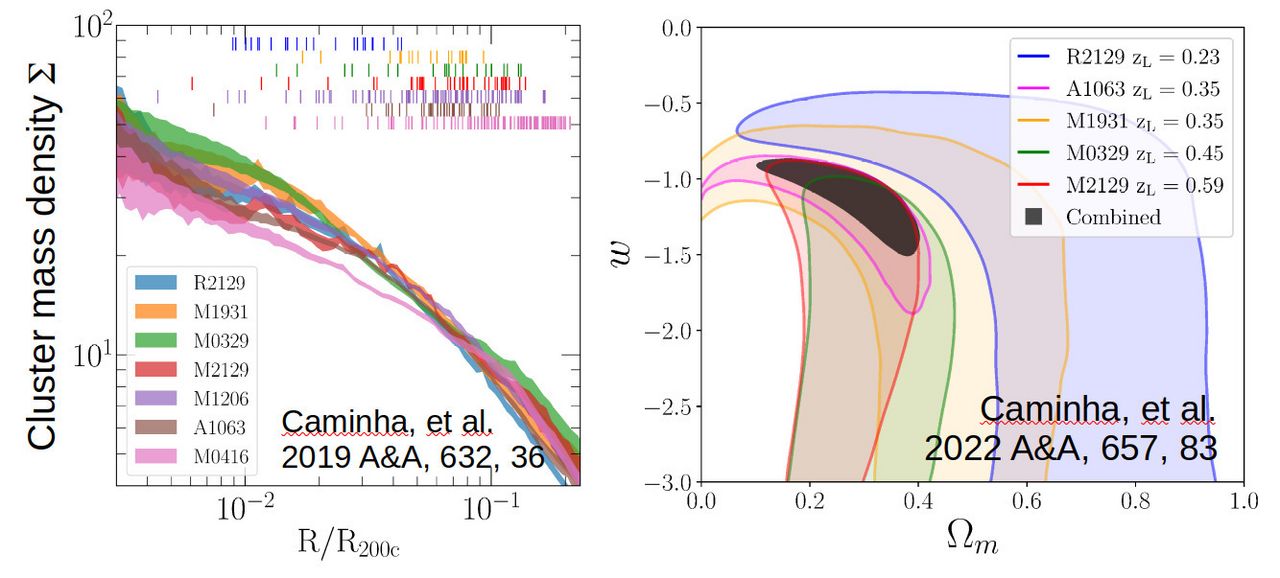
Understanding the mass assembly history of galaxies from the distant to the local Universe is a major research axis in extragalactic astrophysics. Observational and theoretical studies have now established a consistent evolutionary sequence for galaxies in the upper-end of the mass distribution. Massive galaxies have experienced a rapid growth phase during the first 2-3 Gyr after the Big Bang, with major mergers and extraordinary amounts of dust and gas triggering extreme star formation episodes (de Lucia et al. 2006). When the gas reservoirs got depleted, this intense phase of compact, nuclear star formation ceased, leaving red quiescent galaxies (Toft et al. 2007). Their subsequent evolution was driven by minor mergers, mainly with low-mass and gas-poor galaxies, which induced substantial growth in size with moderate increase in stellar mass (Naab et al. 2009). After 8-10 Gyrs, this phase resulted in the massive elliptical galaxies seen in the local Universe.
We are using multi-wavelength observing programs and detailed modeling to address fundamental questions about the physics underlying this evolutionary sequence. In particular, we are using the strong lensing effect to probe: (1) the populations and properties of high-redshift lensed galaxies, and (2) foreground galaxies acting as light deflectors.
First of all, with the boost in sensitivity and angular resolution from strong lensing, we obtain fine diagnostics on the internal properties of galaxies during their initial growth phase. With observations from the optical/near-infrared to the millimeter and radio, we are studying the different components of the interstellar medium (atomic, molecular, and ionized gas, dust content) as well as the stellar populations. We have for instance obtained advanced constraints on the molecular gas phases in these galaxies, including for the dense cloud cores (Cañameras et al. 2018a, 2021), and studied the star formation law down to the small scales of ~100 pc (Figure 1; Cañameras et al. 2017b). By combining these data sets with detailed modeling, we also probe the feedback mechanisms from star formation and/or active galactic nuclei. Recent advances in lensed galaxy reconstruction techniques are also expected to reveal more of their intrinsic morphology (Galan et al. 2021).
Secondly, the strong lensing effect offers a unique chance to obtain robust mass estimates for the foreground lens galaxies, and to gain insights into their baryonic and dark matter components. We are giving a particular focus on the most massive ellipticals residing in overdense environments. For instance, with HST imaging and MUSE spectroscopy, we have shown that joint modeling of strong lensing observables and spatially-resolved stellar kinematics with GLEE (Suyu et al. 2010, 2012) and GLaD softwares (Chirivi et al. 2020, Yildirim et al. 2020) allows us to firmly separate galaxy-scale and extended dark-matter halos (Figure 2; Wang et al. 2022). At the scale of individual galaxies, strong lensing enables the detection of invisible structures such as dark galaxy satellites and complex variations in the mass distribution of the lens (Galan et al. 2022). This opens new avenues to test the influence of the past merging history and the environment on the mass distribution of massive galaxies.
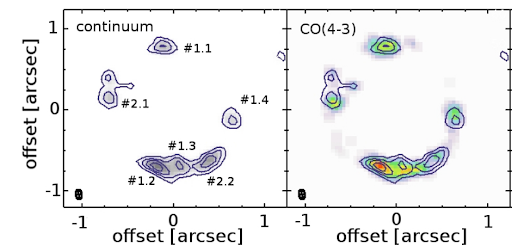
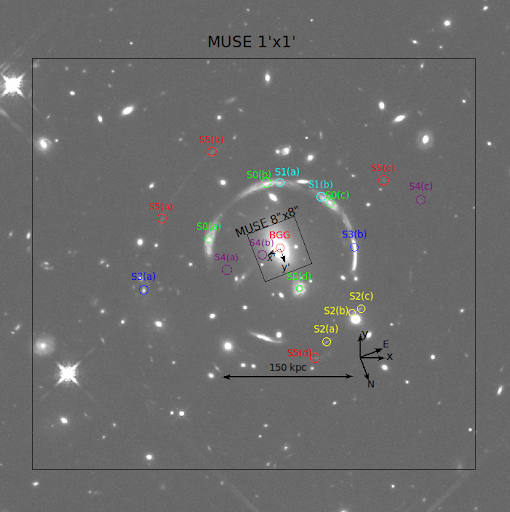
Super-luminous supernovae
Super-luminous supernovae (SLSNe) are 10-100 times brighter than normal SNe, the brightest stellar explosions known to astronomy, which challenge our understanding of how massive stars explode. Probing the physics of these transients can reveal the variety in the final stages of stellar evolution and can offer the tantalising possibility of a new era in the study of the massive stars and galaxy evolution. Their huge luminosity combined with their preference for low-metallicity dwarf host galaxies, make them appealing targets for high-z (young Universe) searches, e.g. with JWST or LSST. We have worked within the ePESSTO+ collaboration and led the Target and Alert Team aiming to find these rare events. We have classified 16 hydrogen-poor SLSNe and 12 hydrogen-rich SLSNe since 2019, and followed up some of them with optical spectroscopy to further investigate their energy source.
Supernova progenitors
Strongly lensed SNe provide excellent opportunities to decipher the progenitors of SNe. Even though Type Ia SNe are a powerful cosmological probe that led the discovery of dark energy (Riess et al. 1998, Perlmutter et al. 1999), their progenitors are still a puzzle after decades of debate. Are they single-degenerate (SD) systems with a white dwarf (WD) accreting material from a non-degenerate companion and exploding when approaching the Chandrasekhar mass limit (e.g., Whelan & Iben 1973), or double-degenerate (DD) systems with two WDs merging (e.g., Tutukov & Yungelson 1981; Iben & Tutukov 1984), or other mechanisms?
Strongly lensed SNe enable observations of a SN explosion right from the beginning, which was nearly impossible to do in the past given both the difficulty of finding SNe at very early phases and the time lag to arrange follow-up observations after a SN is detected. By exploiting the time delay between the multiple SN images, a lensed SN system can be detected based on the first appearing SN image and follow-up (especially spectroscopic) observations of the next appearing SN image can be obtained from the beginning of explosion. Early-phase observations are crucial for understanding the progenitors of SNe.
One of the goals of the HOLISMOKES program (www.holismokes.org) is to acquire such early phase observations of lensed SNe Ia to unveil its progenitors. Through comprehensive microlensing simulations of a suite of SN Ia explosions models, Suyu et al. (2020) showed that microlensing distortion on the early-phase spectra of SNe Ia would typically be negligible, within 1% (1-sigma spread). This provides excellent prospects for acquiring intrinsic early-phase SN Ia spectra through strong lensing to study the progenitors of SNe Ia.
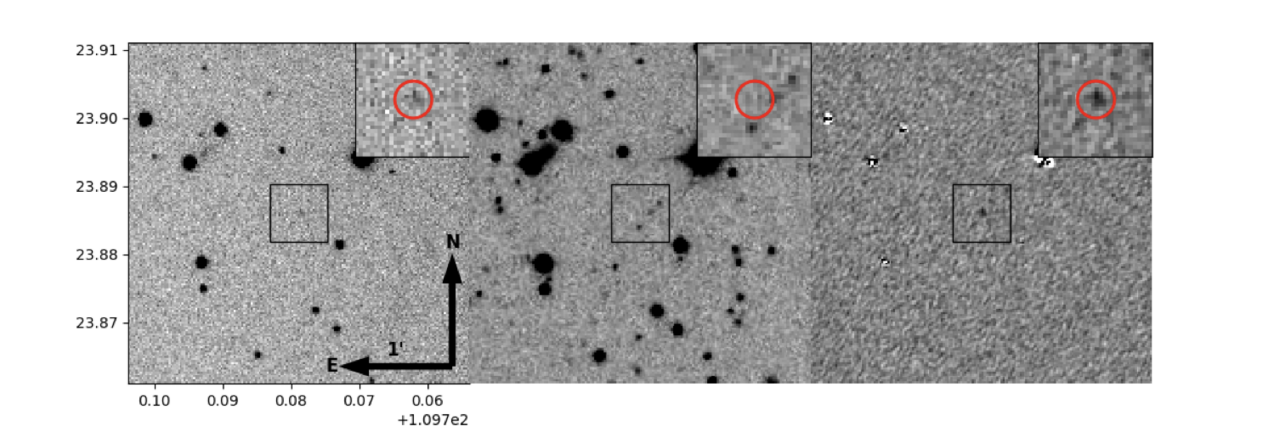
When a star passes sufficiently close to a massive black hole, it can be captured or disrupted. If the star is disrupted outside of the black hole event horizon, known as a tidal disruption event (TDE), some of the stellar material is bound and produces a luminous flare as the stellar debris falls back onto the black hole. These astrophysical transients produce a characteristic light curve that remains observable on the order of a year.
TDEs were a purely theoretical speculation by the time its theoretical model was first proposed in the 1980’s (e.g., Lacy et al. 1982, Hills 1988, Rees 1988). Since then, the events have been detected at an accelerating rate; roughly 100 events are known so far. The number will continue to grow at much faster rates with detections by ongoing (e.g., eROSITA and ZTF) or future (e.g., Rubin Observatory LSST) surveys. In particular, LSST is expected to increase the TDE sample size by a few orders of magnitude (Bricman & Gomboc 2020). Since some of the candidates could be lensed events, it is important to make a reliable estimate for the detection rate of lensed TDEs by future surveys.
We are estimating detection rates of TDEs and strongly lensed TDEs for the upcoming cadenced imaging surveys. The estimate of observable TDEs will depend on the theoretical modeling of the emission mechanism for the TDEs. The comparison between our estimates and the observed rate in the future will place strong constraints on the underlying emission mechanism producing a TDE event which is still debated. In addition, we also plan to use potential microlensing events in strongly lensed TDEs to constrain the size of emission regions in the TDEs. This would allow us to put additional limitations on the emission mechanism in TDEs.
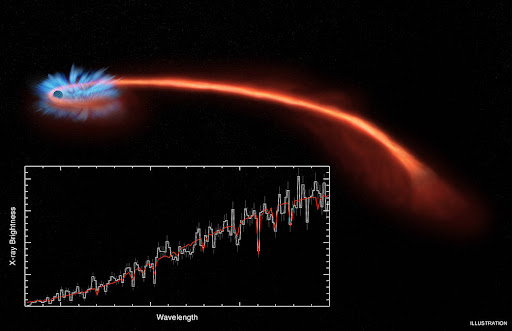
Machine learning techniques have started to play a central role in astronomy, and their utilization will keep growing over the next decades to efficiently analyze the data sets of tera to petabytes from future astronomical surveys.
We are developing supervised machine learning algorithms to identify and model strong gravitational lenses from large data sets, in particular from multiband optical/near-infrared imaging. This involves the development of realistic simulations for training the neural networks. So far, by applying our lens-finding algorithms on existing data from PanSTARRS and HSC surveys, we have identified about 700 new high-quality strong lens candidates (Canameras et al. 2020, 2021, Shu et al. 2022). These new samples are playing a major role for several studies ranging from galaxy evolution to cosmology, and to prepare for LSST and Euclid.
In addition, we use machine learning models like random forests, fully connected neural networks (Figure 1, Huber et al. 2022) and long short-term memory (LSTM) networks (Huber et al. in prep.) to measure time delays in strongly lensed Type Ia supernovae (LSNe Ia) based on their light curves. Especially LSTM networks are very promising to handle a variety of different LSNe Ia expected by the upcoming LSST survey. Our results suggest bias free delay measurements with precisions better than 1 day in a single band if LSNe Ia are followed up with a sufficient cadence and depth.
Deep learning offers impressive performance in many aspects but their results and robustness may be harder to assess compared to more traditional approaches. This limitation can be nicely overcome by explicitly incorporating physical knowledge back into deep learning pipelines. Recent advances in automatic differentiation allowed us to develop such physics-informed analysis pipelines, successfully applied in the context of strong lens modeling (Gu et al 2022, Galan et al. 2022) in preparation for the large data sets available in the near future.
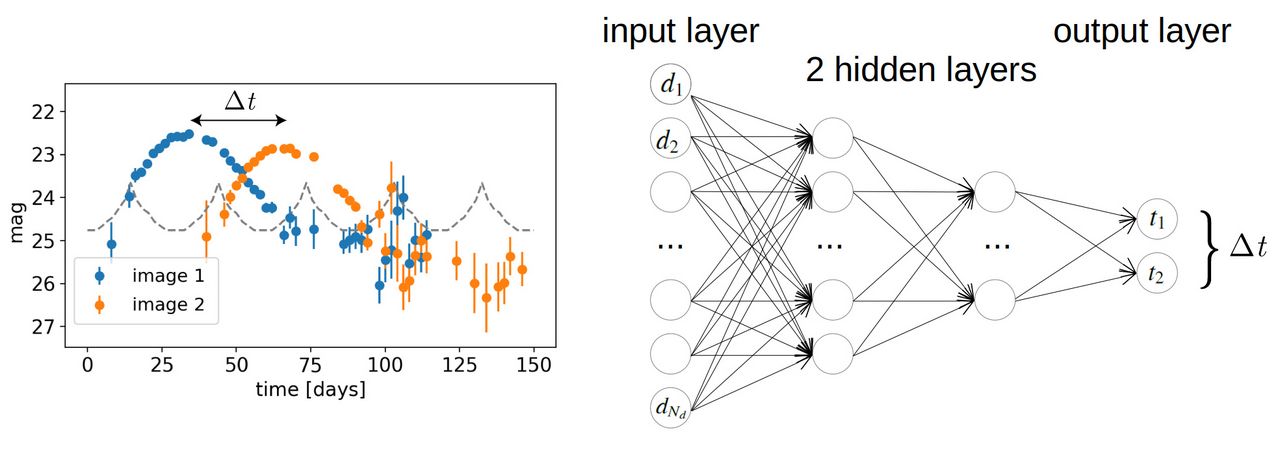